Artykuł sponsorowany
Dlaczego podczas terapii logopedycznej warto korzystać z pomocy naukowych?
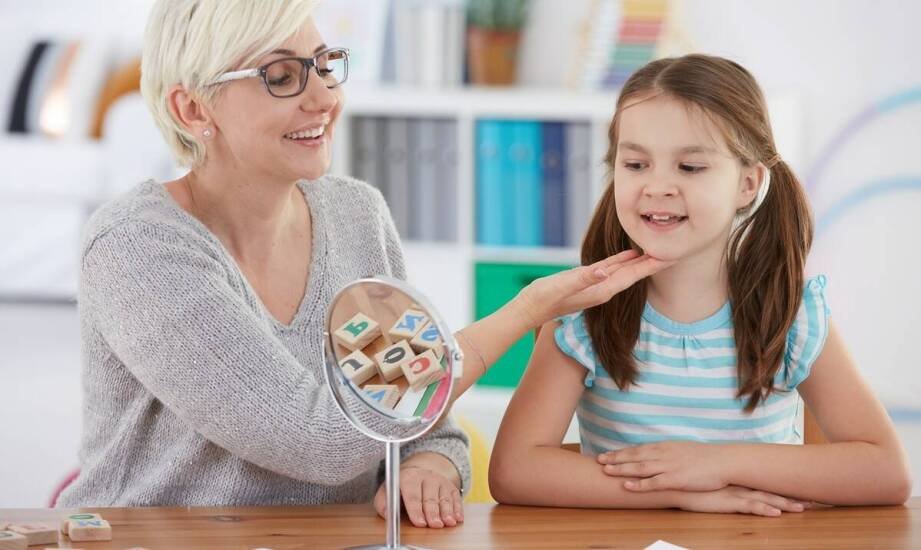
Terapia logopedyczna to proces mający na celu poprawę umiejętności komunikacyjnych osób z zaburzeniami mowy, języka i głosu. Współczesne podejście do terapii logopedycznej opiera się na badaniach naukowych, które dostarczają wartościowych informacji na temat skutecznych metod pracy z pacjentami. Dlaczego warto korzystać z pomocy naukowych podczas terapii logopedycznej? Oto kilka powodów, które przekonują o zaletach takiego podejścia.
Przeczytaj również: Kiedy warto udać się do logopedy z noworodkiem na ocenę wędzidełka?
Ewidencja naukowa potwierdza skuteczność metod
W oparciu o badania naukowe, logopedzi mogą wybrać najbardziej efektywne metody pracy z pacjentami. Dzięki temu proces terapeutyczny jest bardziej celowany i przynosi lepsze rezultaty. Wykorzystanie sprawdzonych naukowo metod pozwala również uniknąć stosowania nieefektywnych technik, które mogą jedynie marnować czas i energię zarówno terapeuty, jak i pacjenta. To z kolei przyczynia się do szybszego osiągnięcia zamierzonych efektów terapii.
Przeczytaj również: Laryngofon medyczny w praktyce logopedycznej: jakie korzyści przynosi jego stosowanie?
Indywidualne podejście do potrzeb pacjenta
Każdy pacjent jest inny i wymaga indywidualnego podejścia. Badania naukowe dostarczają informacji na temat różnorodnych zaburzeń mowy i języka oraz ich przyczyn, co pozwala logopedzie lepiej zrozumieć potrzeby swoich pacjentów. Dzięki temu terapeuta może dostosować metody pracy do konkretnego przypadku i diagnostyki pedagogicznej, co zwiększa szanse na sukces terapii i przyczynia się do poprawy jakości życia pacjenta.
Przeczytaj również: Ile kosztuje detoks narkotykowy i co warto wiedzieć?
Innowacje w terapii logopedycznej
Nauka nie stoi w miejscu, a badania w dziedzinie logopedii prowadzą do odkrywania nowych, innowacyjnych metod terapeutycznych. Korzystanie z najnowszych osiągnięć naukowych pozwala logopedom na ciągłe doskonalenie swoich umiejętności i stosowanie nowoczesnych technik pracy z pacjentami. Dzięki temu terapia logopedyczna staje się coraz bardziej efektywna i skuteczna, co przekłada się na lepsze efekty terapeutyczne. Dodatkowe kursy czy szkolenia specjalisty również potrafią być nieocenionym wsparciem w diagnostyce i leczeniu pacjentów logopedycznych.
Wsparcie dla rodziny i otoczenia pacjenta
Podczas terapii logopedycznej ważne jest również zaangażowanie rodziny i otoczenia pacjenta. Wiedza naukowa dostarcza informacji na temat tego, jak ważne jest wsparcie bliskich w procesie terapeutycznym. Dzięki temu rodzice i opiekunowie mogą aktywnie uczestniczyć w terapii, co przyczynia się do szybszego osiągnięcia zamierzonych efektów. Ponadto, nauka dostarcza praktycznych wskazówek dotyczących tego, jak można wspierać rozwój kompetencji językowych dziecka na co dzień, co zwiększa efektywność terapii.
Podsumowując, korzystanie z pomocy naukowych podczas terapii logopedycznej przynosi wiele korzyści. Ewidencja naukowa potwierdza skuteczność stosowanych metod, pozwala na indywidualne podejście do potrzeb pacjenta, wprowadza innowacje w terapii oraz wspiera zaangażowanie rodziny i otoczenia pacjenta. Dzięki temu terapia logopedyczna staje się coraz bardziej efektywna i skuteczna, co przekłada się na poprawę jakości życia osób z zaburzeniami mowy, języka i głosu, a także ich rodzin i bliskich.